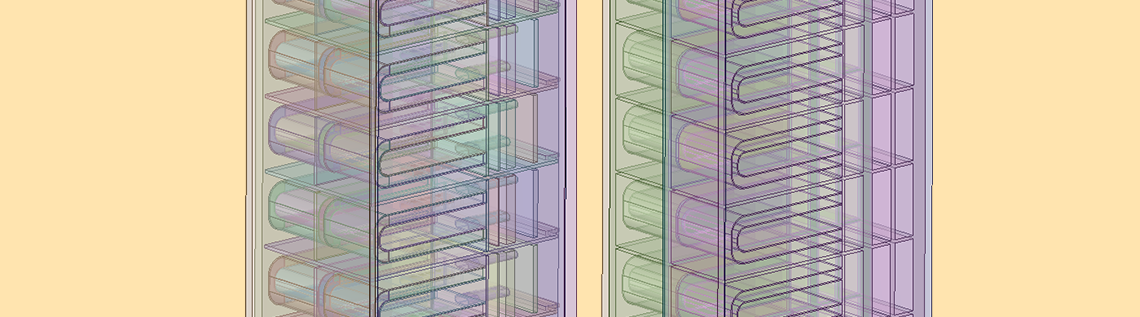
We can solve many of the world’s energy sustainability problems by harnessing the power of the Sun through fusion energy. Tokamaks are devices that test the practicality of employing fusion energy on Earth. ITER, which will be the largest tokamak on Earth when complete, has required years of research and a magnitude of resources. Using COMSOL Multiphysics, a group of researchers investigated whether the tritium breeding modules (TBMs) in ITER produce magnetic perturbation that could generate unacceptable plasma losses.
The ITER Tokamak: Creating Fusion Energy to Better Power the World
When hydrogen atoms collide at high speeds, they fuse into helium and release large amounts of energy. This process, known as thermonuclear fusion, is what powers the Sun and stars and it has the potential to be a sustainable, sufficient, and efficient source of energy for Earth. But how can we harness such power and scale it down for our own use?
Tokamaks are experimental machines used to capture the power of fusion energy. The term comes from a Russian acronym that translates to “a toroidal chamber with magnetic coils”. These devices, which range from extremely large to small enough to fit on a table, generate a magnetic field that is able to confine plasma in the shape of a torus. This whole process causes the same atom collision and fusion that creates fusion power. The components of a tokamak include a large vacuum vessel, superconducting magnets that produce the magnetic field that controls the plasma, blanket modules, a divertor at the bottom of the machine that controls exhaust and waste, and a cryostat that keeps the superconducting magnets cold.
One tokamak in particular, ITER, is tasked with proving fusion energy on Earth is possible and achievable, as well as producing net energy. Net energy is when the total amount of fusion power produced is greater than the amount of energy needed to heat the plasma. ITER will not utilize the energy that is being produced, nor does the input power include the power needed to produce the magnetic field.
A scaled model showing a section of the ITER tokamak. Image by Rama — Own work. Licensed under CC BY-SA 2.0 France, via Wikimedia Commons.
Meaning “the way” in Latin, ITER is a collaborative effort among thousands of scientists. When construction is complete, it will be the world’s largest tokamak device, totaling at 23,000 tons. There are seven main entities invested in constructing and operating ITER — including China, the European Union, India, Japan, Korea, Russia, and the U.S. — but many more are involved in some way, such as Finland, where the researchers discussed in this blog post are based.
Experimental testing for ITER has yet to begin, as building a project of this size requires substantial time, resources, and expertise. The foundation for ITER was completed in August of 2014, and the basement and lower levels of the project are currently under construction. While the time frame for ITER’s completion is years into the future, proving that it is a viable concept can be performed now with the capabilities of simulation.
Evaluating Test Blanket Modules for Tritium Breeding
One of the major goals of the ITER tokamak is to demonstrate the production of tritium within its vacuum vessel. Tritium, an isotope of hydrogen, is required for the operation of ITER, or any tokamak for that matter, to produce fusion energy. Unfortunately, the world’s supply of naturally occurring tritium is limited and there is not enough to supply our energy needs. Fortunately, tritium can be produced inside a tokamak when neutrons escape from the plasma and interact with lithium kept in its blanket structures.
To ensure the success of this process, known as tritium breeding, testing needs to occur in an actual fusion environment. But, as we’ve already discussed, ITER is not yet ready for actual testing. For now, researchers from Aalto University in Finland and Fusion for Energy, the organization responsible for Europe’s contributions to the ITER project, have used COMSOL Multiphysics to test an iteration of the tritium breeding blankets, called test blanket modules (TBMs).
There are six different design iterations of the TBMs, and the researchers here investigated the European design. TBMs are enormous blocks of ferritic material that are magnetized by the strong magnetic field of the ITER tokamak. When the TBMs magnetize, they perturb the magnetic field that confines the plasma for fusion energy. Taina Kurki-Suonio, who leads the research team, notes that when this process happens, it actually looks like fingers are reaching toward the TBMs from the edges of the plasma.
A glimpse of the complex physics behind the ITER tokamak.
The perturbations caused by the magnetization of the TBMs aren’t notably large, but they could possibly deteriorate the confinement of the very energetic ions in the plasma, such as the alpha particles created from the fusion reactions. For the most part, these ions don’t collide, but rather follow the field lines of the magnetic field. For field lines close to the walls of the vessel, fast ions could collide with material structures and slowly erode the wall, or even partially destroy components of the wall. Any small defect or malfunction in ITER could result in expensive and time-consuming repairs. Luckily, the magnetic perturbation of the TBMs, and the resulting deterioration of the plasma confinement and vessel walls that may occur, can be quantified in 3D by numerical studies.
Calculating the Magnetic Field and Vector Potential of the ITER Tokamak
In order to check TBMs to avoid the potential for failure, the researchers aimed to calculate the ITER’s magnetic field and vector potential, which is important to analyze the plasma response. First, they evaluated the magnetization of the ferritic components. This was done by putting all of the current-carrying components, i.e., all of the coils and the plasma current, into COMSOL Multiphysics, along with the FIs and TBMs.
The magnetic field from this calculation would have otherwise been suitable for fast ion studies (for which the fields were intended) but, due to memory limitations, the plasma mesh couldn’t be made as dense as needed. Therefore, the team only took the magnetized ferritic components from the first COMSOL Multiphysics calculation and plugged them into another model with a denser plasma mesh. By superimposing the perturbation field from this latter calculation to the field calculated from the currents in the coils and plasma using only the Biot-Savart law, they were able to find quality fields with contributions from all of the necessary components.
Konsta Särkimäki, another researcher on the ITER simulation team, says that they appreciated using COMSOL Multiphysics for their research because the geometry, meshing, physics, and solvers are all included in the same package. As their team had written MATLAB® script of the entire model from scratch, they also found the LiveLink™ for MATLAB® interfacing capabilities to be very useful for their needs.
The complex components of ITER’s geometry include 18 toroidal field coils that are D-shaped, superconducting, and used to enclose the plasma; 6 each of poloidal and central solenoid field coils; and the current-carrying plasma that is assumed to be toroidally symmetric. The plasma cross section is covered by rectangular mesh. The entire torus, the main shape of the tokamak, is enclosed in a finite sphere of 14 meters and an infinite elements shell.
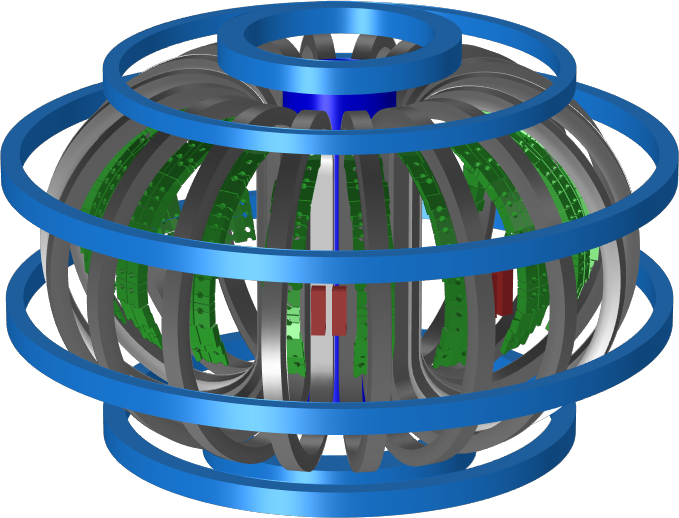
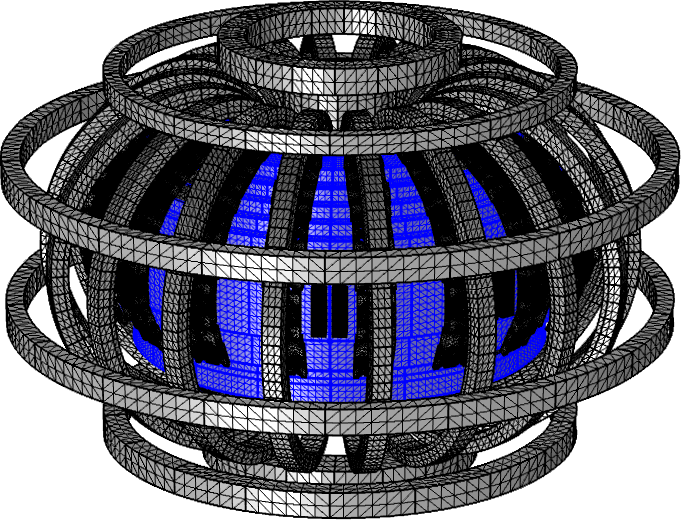
The model geometry of the coils and ferritic components of the ITER tokamak (left) and the meshed geometry, with the plasma shown in blue (right).
The TBMs themselves were modeled after the European helium-cooled pebble bed (HCPB) model. Ferritic inserts (FIs), another component of the geometry, were modeled as ferromagnetic materials along with the TBMs. The purpose of FIs is to reduce the variation in the toroidal field in the tokamak that is caused by having only 18 toroidal field coils.
A meshed TBM geometry (left) and FI models with varying levels of detail (right).
The team removed small details of the model and simplified many of its components because as is, ITER is way too large and detailed for finite element analysis. The research team still ended up with a humongous model with over 200 million degrees of freedom, as well as a computational domain that ranged from a 10-meter radius to elements of only a few millimeters. Even when using the iterative solver in COMSOL Multiphysics, the model required about 800 Gb of RAM and took 3 days to solve with a Finnish supercomputer called Taito, using 32 CPUs.
The geometry of the TBM before (left) and after (right) simplification.
Using the AC/DC Module, the researchers included toroidal and poloidal field coils and toroidal plasma as free currents. They created MATLAB® routines to return the unit vector parallel to the spine curve of the toroidal coils, as well as to calculate the magnitude of the toroidal plasma current density. All of the domains in the model were given the same electrical properties.
As a result of their simulation, the researchers were able to calculate the flux density and vector potential for various operating scenarios of the ITER tokamak. They combined these COMSOL Multiphysics results with the fields obtained using the Biot-Savart law to arrive at the device’s total magnetic field.
Results showing the magnetic flux density and vector potential of the tokamak for the total field (top row) and the field due to the ferromagnetic components (bottom row).
The magnetic field was then decomposed into toroidal Fourier components so that they could be used as input for spectral plasma response codes.
The toroidal Fourier components taken from the calculated magnetic field.
The magnetic fields found from this simulation were verified against earlier work and were found to agree well with one another.
Looking Ahead to the Goals of Fusion Power
The research team performed several studies using the data from their COMSOL Multiphysics model. Such research included a study on the confinement of fast ions and how they are influenced by externally generated magnetic perturbations. Another study concerned how ITER’s plasma responds to these perturbations. Särkimäki notes that the research team generally agrees that the magnetic fields modeled with COMSOL Multiphysics are the most detailed that they have to date. With the help of simulation, our world is one step closer to sustainable fusion power.
Further Reading
- Read the full research paper: S. Äkäslompolo et al, “Calculating the 3D magnetic field of ITER for European TBM studies,” Elsevier B.V., 2016.
- Stay up-to-date on developments and news regarding the ITER tokamak
- Read this blog post to learn about modeling (much more basic) coils in the AC/DC Module
- Got 3 minutes? Watch this video on modeling computational electromagnetics
MATLAB is a registered trademark of The MathWorks, Inc.
Comments (0)